By Javier Vinós & Andy May
Reposted from Climate Etc.
“The atmospheric heat transport on Earth from the Equator to the poles is largely carried out by the mid-latitude storms. However, there is no satisfactory theory to describe this fundamental feature of the Earth’s climate.” Leon Barry, George C. Craig & John Thuburn (2002)
3.1 Introduction
Nearly all the energy that powers the climate system and life on Earth comes from the sun. Incoming solar radiation is estimated at 173,000 TW. By contrast geothermal heat flow from radiogenic decay and primordial heat is estimated at 47 TW, human production of heat at 18 TW, and tidal energy from the Moon and the Sun at 4 TW. Other sources of energy, like solar wind, solar particles, stellar light, moonlight, interplanetary dust, meteorites, or cosmic rays, are negligible. Solar irradiance, thus, constitutes over 99.9 % of the energy input to the climate system.
The energy received from the sun changes over the annual cycle by 6.9 % due to the changing Earth-Sun distance. The Earth is closest to the sun (perihelion) around the 4th of January and farthest (aphelion) around the 4th of July. Although half the Earth is illuminated by the sun at any given time (50.2 % due to the difference in size), the changes in the Earth’s axis orientation towards the sun, the irregular distribution of land masses, changes in albedo, and regional changes in surface and atmosphere temperature, cause important seasonal changes in the amount of reflected solar shortwave radiation (RSR) and outgoing longwave radiation (OLR). As a result, the temperature of the Earth is always changing and the planet is never in energy balance.
Contrary to what could be naively expected, the Earth is warmest just after the June’s solstice, when it is farthest from the sun, and coldest just after the December’s solstice, when it is receiving 6.9 % more energy from the sun. Earth’s average surface temperature is c. 14.5 °C (severe icehouse conditions), but during the year it warms and cools by 3.8 °C (Fig. 3.1). As expected, the Earth emits more energy (total outgoing radiation, TOR) when it is cooling and less when it is warming, regardless of what it is receiving at the time, so the idea of an energy balance at the top of the atmosphere (TOA) is clearly wrong. The Earth displays little interannual temperature variability but there is no reason to think we properly understand the mechanisms involved in Earth’s thermal homeostasis.
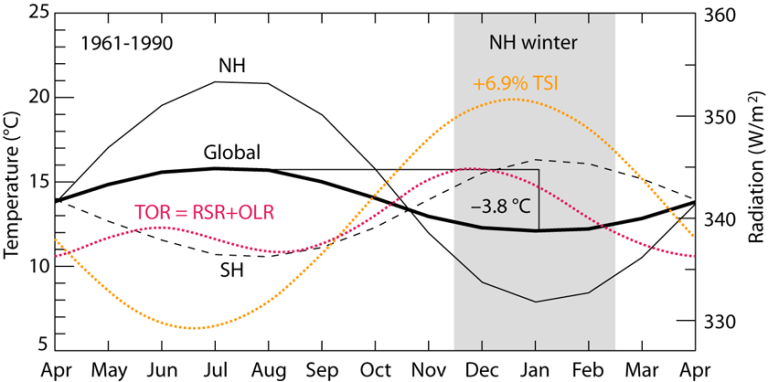
Fig. 3.1. Yearly temperature and radiation change.
The global surface average temperature of the planet (thick line) changes by 3.8 °C over the course of a year, mostly because the NH (thin line) varies by 12 °C. The planet is coldest during the month of January, despite receiving 6.9% more total solar irradiance (TSI, dotted yellow line) in early January when the Earth is in perihelion. The planet has two peaks of energy loss (TOR, Total Outgoing Radiation, outgoing longwave and reflected shortwave, dotted red line) when each hemisphere cools, with the highest during the cooling of the NH. Between November and January, the planet emits more energy (TOR) than at any other time. SH, dashed line. NH winter, light grey area. 1961–1990 temperature data from Jones et al. 1999. Radiation data from Carlson et al. 2019.
What it is clear from figure 3.1 is that although the climate system is entirely powered by solar irradiance, what determines the Earth’s temperature is what the climate system does with that energy, and the climate system is extremely complex. As Barry, et al. (2002) say in the quote at the top of this part, modern climatology lacks a proper theory of how energy is moved within our planet’s climate system. It is possible to model what it is not properly understood, even if very complex, but to believe such a model is foolish.
The energy from the sun comes in a straight line from its surface, as can be clearly appreciated during a total eclipse. The sun has an apparent size of 0.5° of arc in Earth’s sky and is located in the plane of Earth’s solar orbit, called the ecliptic. The ecliptic is the projection of Earth’s orbital plane onto the sky, it is also the path the most vertical rays from the Sun make around the globe, at the local noon, during a 24-hour day. Due to Earth’s axial tilt, the sun is not always directly above the equator, and moves from being above 23.44°N at the June solstice to 23.44°S at the December solstice. The position of the sun at any given daytime determines the angle of incidence of its radiation. At a higher angle of incidence (sun lower on the horizon) the energy arriving from the sun is spread over a larger surface area, decreasing the amount of energy per unit of horizontal area. The flux of solar radiation per unit of horizontal area for a given locality is the solar insolation, and it is higher at solar noon the closer in latitude to the declination of the sun, which marks the position of the ecliptic with respect to the equator. Solar insolation is the most important determinant of local surface temperature.
As a result of the position of the sun with respect to the Earth, most energy enters the climate system in the tropics. However, OLR increases with the absolute temperature of the surface, and decreases with the greenhouse effect, and cloud cover. As the average absolute temperature of the surface does not vary that much with latitude (278–300 K between 60°N–60°S), and greenhouse gas concentration and cloud cover tend to be higher in the tropics, OLR does not vary much with latitude. The result is that the net radiation flux at the TOA is positive (more incoming than outgoing) on the annual average between c. 30°N-30°S and negative between c. 30° and the pole. However, during the Dec-Feb season the net flux is negative north of 15°N (Fig. 3.2), and most of the Northern Hemisphere is losing energy. The resulting cooling from reduced insolation and a net energy deficit creates a latitudinal temperature gradient (LTG). Energy is moved from latitudes where there is a net gain of energy (energy source) to latitudes where there is net loss of energy (energy sink to space), along the LTG (Fig. 3.2), by meridional transport (MT).
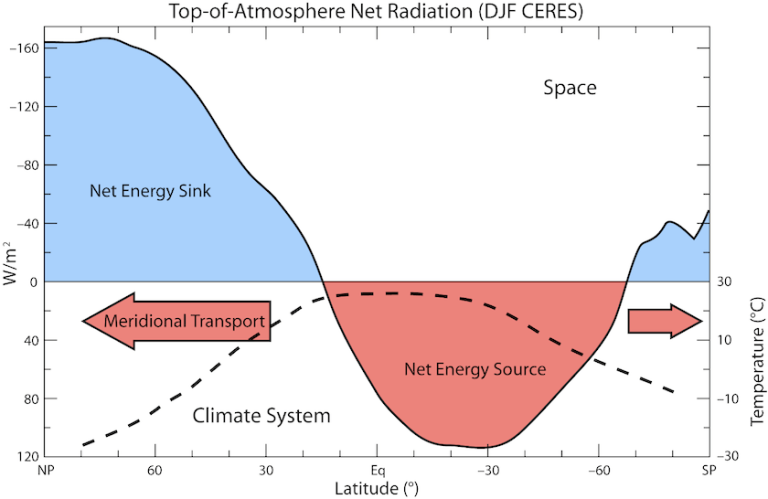
Fig. 3.2. Net radiation flux at the top of the atmosphere for Dec-Feb (Temperature data from Hartmann 1994. Radiation data from Randall 2015).
Positive net flux values (red area in fig. 3.2) indicate a net energy flow into the climate system, and negative values (blue area) indicate a net energy sink, that is, a net flow to space. Areas are not proportional to the amount of energy due to the geometry of the Earth. Meridional transport moves energy, among other things, from regions with an energy surplus to regions with an energy deficit along the gradient in temperature (dashed line, near-surface air temperature for January). Meridional transport moves a lot more energy towards the winter pole.
Without MT, the temperature of the regions where the net flux of energy at the TOA is negative would decrease continuously until OLR emissions are sufficiently low to match insolation. In the polar night regions that temperature would be close to absolute zero (–273.15 °C). MT is carried out by the atmosphere and the ocean along the temperature gradient and is variable over time. It transports a lot more energy (stronger MT) in the winter hemisphere (Fig. 3.2).
3.2 The latitudinal temperature gradient defines the planet’s climate
In the physical universe processes tend to happen spontaneously along gradients, whether they are gradients in mass, energy, or any manifestation of them, like gravity, pressure, or temperature. The Earth’s surface LTG is a direct consequence of the latitudinal insolation gradient. Enthalpy (energy adjusted for volume and pressure) tends to move along the LTG from regions of higher to regions of lower enthalpy. This is the basis of MT, but given the complexity of the climate system, it is far from a passive process that depends only on the temperature difference between the tropics and the poles. Instead, it is a highly regulated process that can drive more energy for a smaller temperature difference and less energy for a larger temperature difference. As will be shown in the next part, MT has increased in the first two decades of the 21st century, despite the Arctic being warmer, reducing the LTG.
We know that the Earth’s LTG has varied a lot over the geological past of the planet. We saw in Part I that Wladimir Köppen, the Russo-German scientist who studied the sun-climate effect in the 19th century, established a climate classification that is still in use with modifications. Climate zones are defined in terms of temperature, precipitation, and their seasonal distribution. Many groups of plants and animals are restricted to a habitat with a narrow range of temperatures; and some geological processes are also temperature dependent. Using this type of information Christopher Scotese has mapped past climate history with his Paleomap Project(1). The information thus obtained allows him to geographically reconstruct half a dozen climate zones every few million years, and from that to reconstruct the changing LTG of the Earth’s past. Scotese et al. (2021) defines the climate and global temperatures of each period based on their LTG, demonstrating that it is a fundamental climate variable. Scotese defines the present (21st century) LTG and global temperature as severe icehouse conditions, as demonstrated by the massive permanent ice sheets over Antarctica and Greenland.
The existence of very different past climates of the Earth creates an unsurmountable problem for modern climatology. During the last glacial maximum (LGM), 20,000 years ago, the energy received from the sun was the same as now. Not only that, but the precession and obliquity values were the same as now, and the orbital eccentricity was very similar. The distribution of solar energy over the Earth and the latitudinal insolation gradient were nearly identical to now, yet the climate was very different. Energy input to the climate system must have been lower, because albedo was higher and the greenhouse effect lower. A lower energy input and a larger LTG ought to have drained the tropics of heat via a much stronger MT, but that was not the case. There is still controversy about tropical temperatures during the LGM, but it appears that they were only 1–2 °C colder than present (Annan & Hargreaves 2015). This is consistent with evidence presented by Scotese et al. (2021) that tropical temperatures have not changed much over the course of the past 540 million years despite huge changes in the average temperature of the planet (9–30 °C).
If the LGM creates a problem for how MT operates during a glacial period, the equable climate of the early Eocene results in a paradox that modern climatology cannot solve. Currently the Earth is in a severe icehouse climate with a very steep LTG. Temperature falls by 0.6–1 °C/°latitude from the equator to the winter pole. Such cold or colder conditions as of today have been relatively rare during the past 540 Myr (less than 10 % of the time). The early Eocene Earth had an average temperature estimated at 23.8 °C, that Scotese describes as hothouse conditions. The early Eocene LTG was very shallow, at 0.25–0.45 °C/°latitude, with temperatures at the North Pole above freezing all year round, as attested by the presence of frost-intolerant biota. These hothouse conditions have been even rarer. Over 80 % of the Phanerozoic Eon the Earth had an average temperature of 17–20 °C (Scotese et al. 2021).
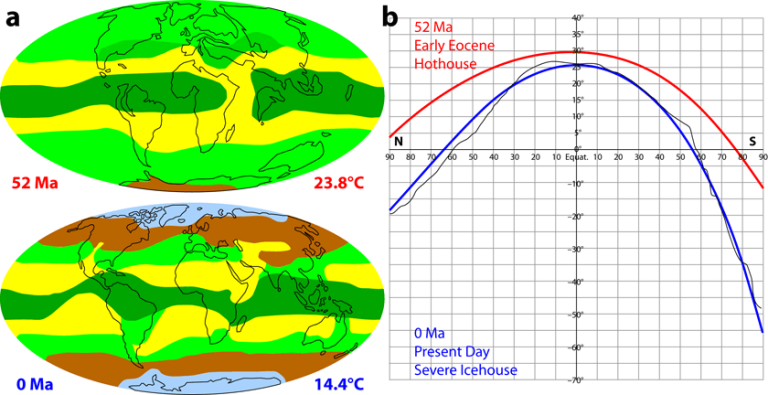
Fig. 3.3. The Earth’s climate is defined by its latitudinal temperature gradient.
a) Climatic belts of the early Eocene hothouse (top) deduced from fossil and geochemical evidence by Scotese et al. 2021, and the present severe icehouse (bottom). Equatorial wet (dark green), subtropical arid (yellow), warm temperate (light green), cool temperate (brown) and polar (light blue) belts. Temperature is the estimated global mean average. b) Latitudinal temperature gradient inferred for the early Eocene (red) and the present (blue) versus measured (black, fine line). After Scotese et al. 2021
The climate of the early Eocene, the Cretaceous, and early Paleogene, is defined as equable, characterized by a warm world with reduced LTG and low seasonality. The failure of modern climate theory to explain these periods has been termed the “equable climate problem” (Huber & Caballero 2011). To reproduce the early Eocene warm continental interior temperatures and above freezing winter high latitudes, models have to raise CO2 levels to 4700 ppm and tropical temperatures to 35 °C. However, the best CO2 estimates for the early Eocene climatic optimum (Beerling & Royer 2011; Steinthorsdottir et al. 2019) place CO2 levels at 500–1000 ppm, and it is unclear that a tropical temperature above 30 °C is possible. The survivability wet-bulb temperature limit for mammals is 35 °C, at which point they become unable to lose heat (Sherwood & Huber 2010). The highest wet-bulb temperature on Earth today is 30 °C, and there is no reason to think that it has been higher at any time in the past at places where mammal fossils are found.
At the root of the equable climate problem lies the “low gradient paradox” (Huber & Caballero 2011). Conceptually, we believe that to have warm poles more heat must be transported there, to compensate for the insolation deficit. Heat MT is a very important part of the planetary energy budget, and it is generally believed that without it the poles would be much colder. But MT depends on the LTG since much of the poleward transport in the present climate is through atmospheric eddies resulting from baroclinic (where temperature gradients exist at constant pressure surfaces) instability. The paradox arises because, counterintuitively, the warm poles of the early Eocene and their much shallower LTG imply a reduced MT. It is no wonder that climate models have such a problem reproducing it. In Part VI a possible solution to the paradox will be offered.
3.3 Meridional transport is mainly carried out by the atmosphere
The lower atmosphere is a thin film of gas, just 1/600 of the Earth diameter (c. 10 km), that has the crucial role of always maintaining a land surface temperature compatible with complex life, something it has done for at least the past 540 Myr. To do that it has to compensate for surface temperature differences arising from differences in insolation. First, it must compensate the difference between day and night. It does so mainly through the greenhouse effect that reduces night cooling, and through the effect of clouds, that increase albedo during the day and reduce night cooling. Then, it must compensate for the latitudinal decrease in insolation and its seasonal changes due to the axial tilt of the planet. It does so through meridional heat transport.
Of these three factors responsible for Earth’s thermal homeostasis, greenhouse effect, clouds, and MT, modern climatology has focused exclusively on the first, developing the CO2 “control knob” climate hypothesis (Lacis et al. 2010). The effect of clouds and their variability on climate change is still largely unknown. With respect to MT, and as figure 3.2 suggests, energy is only exchanged between the climate system and the outside through the TOA, this results in MT necessarily having a net zero value when integrated over the climate system. Moving energy from one region to another does not alter the amount of energy within the system. This fact has resulted in the general belief that changes in MT cannot constitute a significant cause for climate change, producing the most fundamental mistake of modern climatology.
The atmosphere has the outstanding capacity of moving a great amount of energy, fast and efficiently, over the entire surface of the Earth. As a result, MT is carried out mainly by the atmosphere. Only within the deep tropics (10°S–10°N) the atmosphere is inadequate for MT requirements. This is the region where most energy enters the climate system (Fig. 3.4 black dashed line). But the Hadley cell’s upper branch transports dry static heat (sensible + geopotential; Fig. 3.4 red dotted line) poleward, and this is partly compensated for by the lower branch’s equatorward transport of latent heat (Fig. 3.4 red dashed line). Due to this, the ocean must carry out most of the heat transport in the deep tropics. However, the ocean is less efficient at transporting heat than the atmosphere and the energy transport required in the tropics is very large, particularly in the Pacific, due to its size. ENSO is the answer to this problem, as El Niño is the way to periodically transport out of the deep tropics the excess accumulated heat that the regular MT cannot carry. ENSO is part of the global MT system.
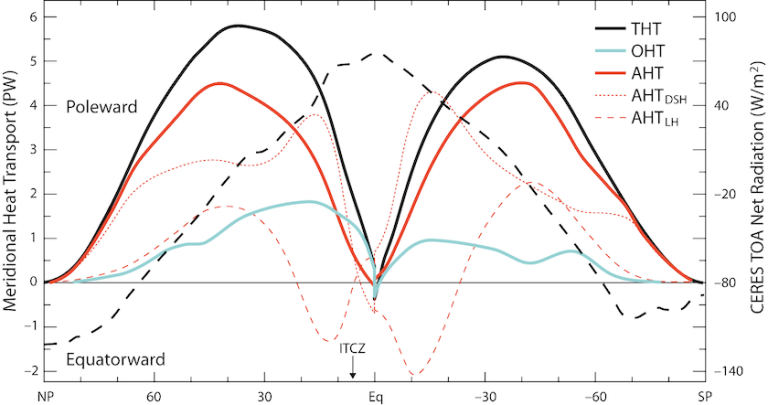
Fig. 3.4. Meridional transport decomposition.
Left, meridional transport in peta Watts calculated from velocity-potential temperature fields and represented as poleward in positive values. THT, total heat transport; OHT, oceanic heat transport; AHT, atmospheric heat transport; DSH, dry static heat (sensible + geopotential); LH, latent heat; ITCZ, inter-tropical convergence zone. After Yang et al. 2015. Right, black dashed line, CERES TOA net radiation flux in Watts/m2, positive is net inflow, or warming. After Randall 2015.
Once outside of the Hadley cell reach, the ocean transfers most of the energy it transports to the atmosphere, particularly at the western ocean basin boundary currents in the mid-latitudes, and poleward latent heat atmospheric transport becomes important. In summary, most of the energy enters the climate system at the photic layer of the tropical oceans, it is then transported outside the deep tropics mostly by the oceans and ENSO, and most of the energy is then transferred to the atmosphere that does the bulk of the transport in the middle and high latitudes. Once the sea-ice edge is reached, the transport is essentially carried out exclusively by the atmosphere, as the energy flux through the sea ice is much less than from the liquid ocean surface. Excluding solar radiation, the rest of the energy flux across the sea surface is positive towards the atmosphere nearly everywhere at every time, except for some high latitude regions during the summer (Yu & Weller 2007). Sea-surface temperature is not as important for ocean-atmosphere energy flux as wind speed and air moisture, the principal factors governing evaporation.
Figure 3.4 shows that MT is asymmetric. Poleward transport at the equator line is near zero, with a small inter-hemispheric transport (0.2 PW northward). The position of the inter-tropical convergence zone (ITCZ, the climatic equator that separates the North and South Hadley cells), varies between 15°S and 30°N, and has an annual mean position c. 6°N. Poleward transport increases with distance from the equator as heat from a bigger region is transported poleward. Northern Hemisphere (NH) MT is bigger because northern oceanic MT is bigger. This is due to a northward inter-hemispheric ocean MT of 0.4 PW, mainly through the Atlantic Ocean, compensated in part by a southward inter-hemispheric MT of 0.2 PW by the atmosphere from the ITCZ (Marshall et al. 2013). Poleward of 45° the northern atmospheric MT becomes larger than the southern, due to a larger sensible heat transport by eddies, particularly during winter. This transport reflects a larger ocean-atmosphere flux at the western boundary mid-latitude currents (Yu & Weller 2007), that is responsible for a warmer winter climate in the European mid-latitudes and for Arctic winter warming. As we can also see in figure 3.4, 70–90° TOA net radiation is more negative in the Arctic than in Antarctica. This is the obvious result of transporting more heat to the Arctic in winter.
Transport of energy by the atmosphere is linked to the transport of mass, momentum, chemicals, moisture, and clouds. It takes place in the troposphere, mainly along preferred routes over ocean basins, and in the stratosphere. As we saw in section 2.5, angular momentum is exchanged between the solid Earth–ocean and the atmosphere. In low latitudes, surface winds are easterly and flow in the opposite direction to the rotation of the Earth, so the atmosphere gains momentum through friction with the solid Earth–ocean that reduces its speed of rotation, while in middle latitudes surface winds are westerly and the atmosphere loses momentum to the solid Earth–ocean that increases its speed of rotation, so a poleward atmospheric flux of angular momentum is required to conserve momentum and maintain the speed of rotation.
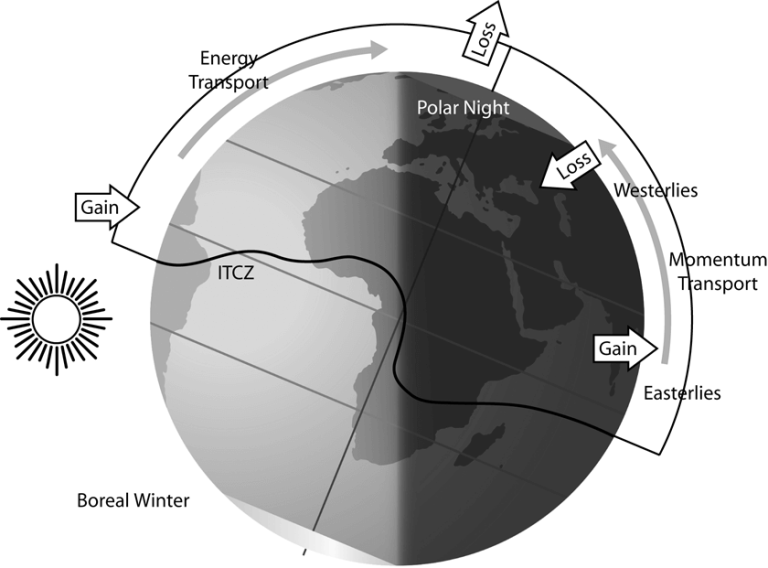
Fig. 3.5. Meridional transport of energy (left) and angular momentum (right) implied by the observed state of the atmosphere.
In the energy budget there is a net radiative gain in the tropics and a net loss at high latitudes; to balance the energy budget at each latitude, a poleward energy flux is implied. In the angular momentum budget, the atmosphere gains angular momentum in low latitudes due to easterly surface winds and loses it in the middle latitudes due to westerly surface winds. A poleward atmospheric flux of angular momentum is implied. Meridional transport of energy and momentum is known to be modulated by ENSO, the quasi-biennial oscillation and solar activity. After Marshall & Plumb 2008
Changes in the atmospheric angular momentum (AAM) must be balanced by changes in the speed of rotation of the solid Earth–ocean to preserve momentum, and they are mostly due to the seasonal changes in the zonal wind circulation. Zonal wind circulation is stronger in winter, when more angular momentum resides in the atmosphere due to a deeper LTG, so the Earth rotates faster in January and July, and slower in April and October, when zonal circulation is weaker. As mentioned in Part II, these small changes in the rate of rotation of the Earth are measured as micro-second changes in the length-of-day (∆LOD), the difference between the duration of the day and 86,400 Standard International seconds. Seasonal variation in ∆LOD reflects changes in zonal circulation (Lambeck & Cazennave 1973) and, therefore, in MT. The biennial component of ∆LOD reflects changes in the QBO (Lambeck & Hopgood 1981), the 3–4-year component matches the ENSO signal (Haas & Scherneck 2004), and the decadal change in ∆LOD reflects changes in solar activity (Barlyaeva et al. 2014).
The Sun, QBO and ENSO constitute three factors modulating the coupling of the tropical stratosphere to the polar vortex (PV) and the polar troposphere, regulating heat and moisture transport to the winter pole. Since they affect the zonal wind circulation it is not surprising to see they also affect the speed of rotation. But while the role of ENSO and the QBO in changing the AAM and ∆LOD is widely known and reported, the role of the sun remains largely ignored.
3.4 Winter transport to the Arctic. The biggest heat-sink of the planet
It is believed that the hemispheric difference in temperature (Fig. 3.1) is due mainly to the larger land fraction in the NH (67.3 % of global landmass) that warms and cools more than the ocean surface. The answer is however more complex, as it also involves the asymmetry in MT (Kang et al. 2015). As we have seen, some of its consequences are the preferential location of the ITCZ in the NH, and a net inter-hemispheric heat transport from the SH to the NH. Hemispheric transport asymmetry results also from the reduction in MT to the South Polar Cap, hindered by the Antarctic Circumpolar Current and the Southern Annular Mode, that climatically isolate Antarctica. The result from these asymmetries is that despite the South Pole being much colder, more energy is transported to the North Pole (Peixoto & Oort, 1992). As a result of its warmer atmosphere, the 70–90°N polar region loses c. 10 W/m2 more heat over the year than the 70–90°S polar region. The loss is much bigger during the boreal winter, when the atmosphere transports 120 W/m2 across 70°N, than during the summer, when it transports 80 W/m2 (Peixoto & Oort, 1992). Most of the transport is carried out by transient eddies and the mean meridional circulation, but the winter-summer difference is mostly due to stationary eddies along storm tracks that in winter are responsible for most of the increase (Fig. 3.6). Over 80 % of the energy transported during the warm season to the north polar region is used to melt snow and ice, and warm the ocean. About two thirds of that energy constitutes energy storage that is returned to the atmosphere during the cold season cooling and re-freezing, and mostly lost through OLR. As a result of these differences, the north polar region loses 20 % more energy than the south polar region during the respective winters, constituting the biggest heat-sink of the planet (Fig. 3.2).
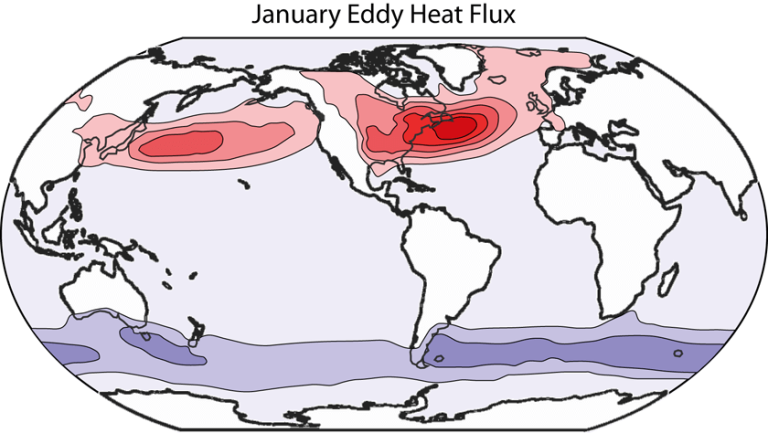
Fig. 3.6. January northward heat flux by eddies.
During boreal winter the NH subtropical jet has two maxima downstream of the Himalaya and Rocky Mountains over the Pacific and Atlantic oceans, respectively. These wind speed maxima result in vigorous mid–latitude cyclones following storm tracks that define the main gateways into the Arctic. Contour is 5 K m/s. Blue shading in the SH indicates southward flux. After Hartmann 2016
During winter, nearly all the energy lost at this heat-sink is transported there by the atmosphere, as the equilibrium temperature of sea water in contact with ice is practically constant regardless of the atmospheric temperature and sea-ice thickness. Sea-ice constitutes a very good insulator (K ≈ 2.2 W/m K). Compared to a loss of 310 W/m2 for exposed waters at a 30 °C temperature difference, a 2 m thick ice layer reduces the loss to only 30 W/m2 (Peixoto & Oort, 1992). It is clear that the great loss of winter sea-ice for the past 45 years constitutes a strong negative feedback on global warming.
Dry static (sensible + geopotential) heat is brought into the winter Arctic by both the middle (20–100 km height) and lower atmosphere, while latent heat (moisture) is transported almost exclusively by the lower atmosphere. Figure 3.7 shows NH winter atmospheric heat transport. Upper atmosphere transport is inter-hemispheric; however, it involves only 0.1 % of the atmosphere mass, making it irrelevant for energy considerations. The stratosphere contains 15% of the atmospheric mass, and its meridional transport is termed the Brewer–Dobson circulation (BDC). Air enters the stratosphere at the tropical pipe (Fig. 3.7), through a cold region above the tropical tropopause where it loses most of its water vapor. In the upper stratosphere the deep branch of the BDC is inter-hemispheric and moves toward the winter pole. In the lower stratosphere, the shallow branch of the BDC has a poleward direction, although it is stronger towards the winter pole. In the middle and high latitudes, the BDC air descends through the tropopause toward the surface. The BDC takes place through a meridional wind thermal balance established by the LTG and is powered by planetary and synoptic waves that release energy and momentum to the mean flow when they dissipate.
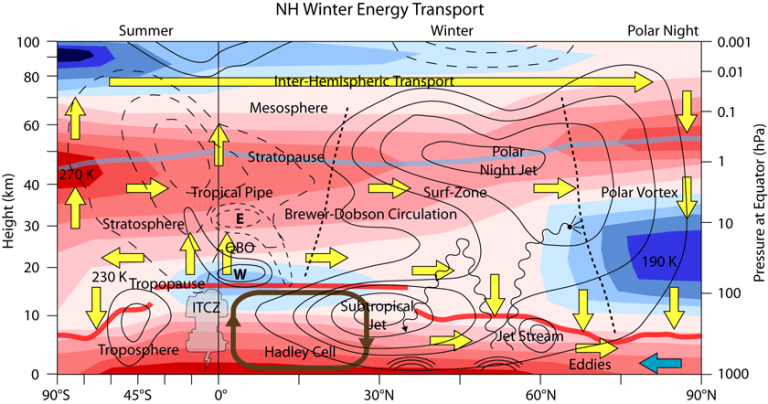
Fig. 3.7. Schematic of atmospheric circulation at the December solstice in a two-dimensional lower and middle atmospheric view.
Background colors indicate relative temperatures at 10 K steps, with red being warmer and dark blue being cooler. Vertical scale is logarithmic, and the SH latitudinal scale is compressed. Westerly winds represented by thin lines; easterly winds by thin dashed lines. The tropopause (thick orange line) separates the troposphere and stratosphere, and the stratopause (thick steel blue line) the stratosphere and the mesosphere. Thick dotted lines separate the tropical pipe (ascent zone), the surf-zone (wave-breaking zone), and the polar vortex. Planetary waves (undulating lines) generate at areas of contrast (concentric lines at surface) and can pass through the stratosphere, be deflected and break at the stratosphere or be refracted back to the troposphere. The quasi-biennial oscillation (QBO) is shown with its easterly and westerly components close to the Equator. The intertropical convergence zone (ITCZ) is shown as a tall stormy cloud. The Hadley circulation is displayed in dark brown. Other atmospheric circulation is represented by yellow arrows except the lower tropospheric equatorward circulation in turquoise. The stratospheric circulation is termed the Brewer–Dobson circulation. Its deep branch (upper stratospheric) and mesospheric circulation are inter-hemispheric from the summer to the winter pole. Tropospheric circulation is carried out mainly by eddies, and the rest by the mean residual circulation. At the December solstice, regions North of 72° are in polar night. From Vinós 2022
The autumn cooling of the Arctic atmosphere causes the end of the summer polar anticyclone, as the pressure decreases and the easterly winds that prevent upward wave propagation are replaced by westerly winds. A pole-centered cyclone (low pressure center with anti-clockwise rotating winds), known as the polar vortex (PV) forms then. The winter westerly winds of the NH are so strong that they only allow vertical wave propagation to the stratosphere of planetary waves of the highest amplitude (zonal wavenumber 1 and 2). The waves release their momentum and energy in an area of the stratosphere known as the “surf-zone” (McIntyre & Palmer 1984). The effect on the zonal mean circulation is a deceleration of westerly winds disrupting the thermal structure. As the LTG cannot be maintained under weaker westerly winds, air is forced down inside the PV, warming adiabatically, and up outside the PV, cooling. The Arctic polar atmosphere can warm by 30 °C in the lower stratosphere and up to 100 °C in the upper stratosphere. Afterwards, as the Arctic atmosphere is under strong radiative cooling during the winter, the stratosphere cools and the westerlies regain speed. When wave propagation weakens, the opposite happens and temperature at 30 km above the Arctic can become as low as –80 °C.
Northward of 20°N the atmosphere becomes the main carrier of heat poleward. During the NH winter, heat is transported to the Arctic mainly by stationary eddies (planetary waves) and transient eddies (cyclones). Cyclones preferentially generate, propagate and dissipate in storm tracks and tend to form where surface temperature gradients are large (Shaw et al. 2016). The jet stream influences their speed and direction of travel. The winter eddy heat flux reveals the preferred storm track areas (Fig. 3.6; Hartmann 2016).
A few extreme events per season associated with individual weather systems are responsible for a large part of the heat and moisture transported into the Arctic winter. Large-scale atmospheric blocking conditions deflect cyclone tracks poleward, and figure 3.8 shows one of these extreme events that took place in the last days of 1999 and first days of 2000, a case studied by Woods and Caballero (2016).
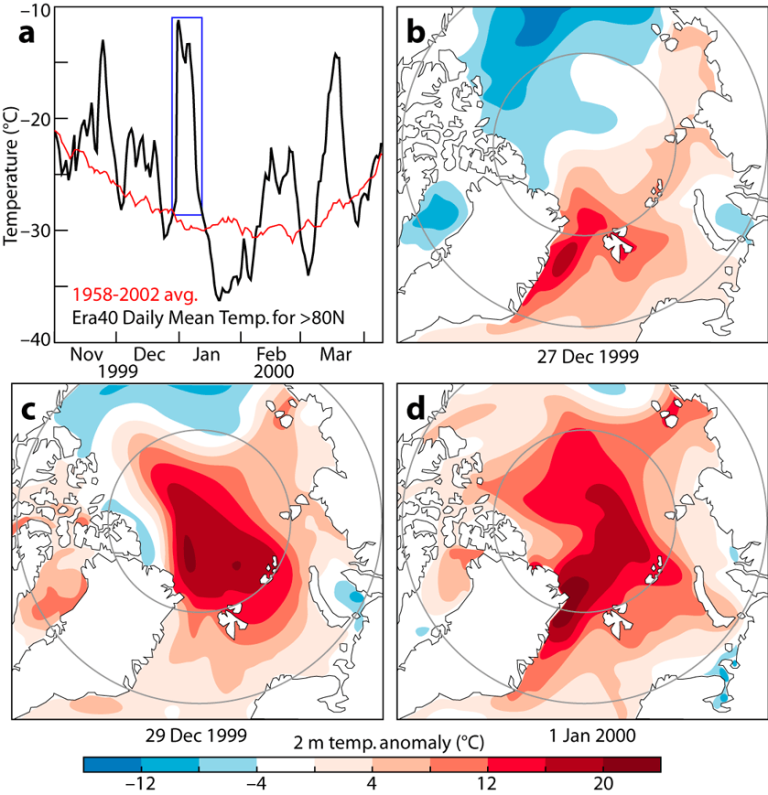
Fig. 3.8. Intense intrusion event of moist warm air into the Arctic in winter.
a) Daily mean temperature North of 80°N for Nov 1999–Mar 2000 (black line) from ERA40 reanalysis, and the 1958–2002 average (red line). A blue rectangle marks the event. Data from the Danish Meteorological Institute (2021). b–d) Surface air temperature anomaly in the Arctic at different times during the intrusion event. After Woods & Caballero (2016)
According to Nakamura and Huang (2018) blocking develops like a traffic jam when the jet stream capacity for the flux of wave activity (a measure of meandering) is exceeded. Large-scale blocking conditions develop to the east of each ocean basin, deflecting midlatitude cyclones poleward (Woods et al., 2013). As a consequence, a great part of the latent heat transported into the Arctic is the result of a limited number of weather systems that enter the Arctic mainly through a North Atlantic gateway (300–60°E), followed in importance by a North Pacific gateway (150–230°E), and a less important Siberian one (60–130°E; Mewes & Jacobi 2019; Woods et al. 2013). Over the Atlantic, winter blocking strongly anti-correlates with the North Atlantic Oscillation (Wazneh et al., 2021).
Knowing how heat is transported into the Arctic allows us to examine the phenomenon of Arctic amplification. General circulation models have been predicting polar amplification as a result of global warming since their beginnings. After all, as seen in figure 3.3, as the climate of the Earth changes the change in temperature is larger the higher the latitude. However, in modern global warming Antarctic amplification has not been observed, and by 1995 so little Arctic amplification had been observed despite intense global warming the previous 20 years, that Curry et al. (1996) said: “The relative lack of observed warming and relatively small ice retreat may indicate that GCMs are overemphasizing the sensitivity of climate to high-latitude processes.” That was about to change that year when Arctic amplification suddenly accelerated (Fig. 3.9). But the question is still valid. Why was Arctic amplification small before 1996, when intense global warming was taking place, and large after 1996 when global warming rate decreased (the pause)? Modern climatology does not have an answer to that.
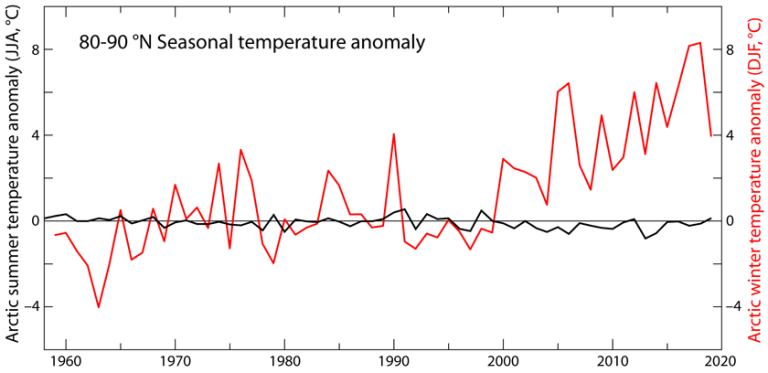
Fig. 3.9. Arctic seasonal temperature anomaly.
Black curve, summer (June–August) mean temperature anomaly calculated from the operational atmosphere model at the European Center for Medium-range Weather Forecast (ECMWF) for the +80°N region. Red curve, the corresponding winter (December–February) mean temperature anomaly for the same region. Reference climate is ECMWF– ERA40 reanalysis model for 1958– 2002. Data from the Danish Meteorological Institute.
As we have seen above (e.g., Fig. 3.2), the Arctic in winter constitutes the biggest heat-sink (net energy loss to space) in the planet. Arctic precipitable water is c. 1.5 cm in summer, but in winter it drops to c. 0.2 cm (Wang & Key, 2005), the lowest value outside Antarctica. As a result, cloud cover becomes lower in winter increasing the energy loss. With a reduced cloud cover, almost no water vapor, and no albedo effect, the Arctic in winter has essentially no feedbacks to the greenhouse effect from CO2. Even more, van Wijngaarden & Happer (2020), note that “the relatively warm greenhouse-gas molecules in the atmosphere above the cold surface cause the Earth to radiate more heat to space from the poles than it could without greenhouse gases.”
It is clear that Arctic amplification is the consequence of an increase in MT, as the Arctic has a negative annual energy budget and the increase in greenhouse effect does not make it less negative. The warming in the Arctic, particularly during the winter, can only come from an increase in the heat transported from lower latitudes. The increase in Arctic heat transport that is not exported back to lower latitudes is distributed between increased OLR and increased downward longwave radiation. The enhanced downward radiation increases surface temperature, but due to the low thermal conductivity of ice, and since the heat flux always goes from the warmer ocean to the atmosphere during winter, temperature inversions commonly result, often accompanied by humidity inversions, and the radiative cooling continues from the top of the inversion or the top of the clouds until the water vapor freezes and precipitates, restoring the original very cold condition (Fig. 3.8a).
Arctic winter heat transport is enhanced at times when high pressure conditions prevail over the pole leading to a weak or split vortex. Warm air then enters the central Arctic ascending over the cold air (isentropic lifting), pushing it outwards. As a result, cold Arctic air masses then move over the mid–latitude continents producing anomalously cold temperatures and snow. Since Arctic amplification started, the frequency of mid-latitude cold winters has increased, something that models cannot explain (Cohen et al. 2020), but something similar took place between 1920–40 (Chen et al. 2018).
In this part we have reviewed how the LTG constitutes the most fundamental climate variable, and the mechanisms by which it drives the MT of energy towards the poles. In the next part we will review what happens when those mechanisms change in a coordinated way, as it happened when Arctic amplification started after 1996.
(1) http://www.scotese.com/climate.htm
References